Severe neonatal hyperbilirubinemia and the brain: the old but still evolving story
A review on the bilirubin catabolism with special emphasis on the neonatal age
Unconjugated bilirubin (UCB) is the yellow pigment of heme catabolism responsible for icterus. Daily formed mainly from the degradation of senescent red blood cells in the spleen, UCB flows to the liver through the blood bound to serum albumin (see Figure 1). After entering the liver mainly by the active transport operated by the organic anion transport polypeptide (OATP) 1B1 and 1B3 (1,2), UCB is transformed by the uridine 5'-diphospho-glucuronosyltransferase 1A1 (UGT1A1) in the water-soluble conjugated bilirubin (CB), thereafter excreted from the body after further biotransformation powered by the intestinal flora (3). This degradation pathway balances bilirubin formation maintaining the total serum bilirubin (TSB: UCB + CB) level in a physiological range of 0.2–1 mg/dL (3.4–17.4 µmol/L), with UCB being the predominant fraction. When blood UCB level exceeds the serum albumin binding capability, the unbound portion of the pigment [free bilirubin (Bf), less than 0.1% in physiological conditions (4,5)] enters the tissues leading to the yellow coloration of the skin, the so-called icterus (from the Greek = yellow, or jaundice from the French “jaune” = yellow). Icterus is common in neonates, and mainly due to (see Table 1): (I) the increased red blood cell turnover occurring after birth (6,35,36); (II) the undeveloped UGT1A1 activity (6,7,9); and (III) the quite total absence of the intestinal flora in neonates (11-13).
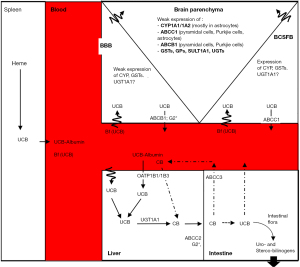
Table 1
Variable | Pre-term | Full-term | Ref. |
---|---|---|---|
Heme production (80% senescent red blood cells, 20% ineffective erythropoiesis + myoglobin and cytochromes) | Increased red blood cell fragility (vs. full-term) | Increased red blood cell turnover (vs. adult) | (3,6,7) |
OATP1B1/1B3 (liver) | No data | 0.2% vs. adult. In 1 year-old baby still only 1% of adult expression | (8) |
UGT1A1 (liver) | Increased immaturity (vs. full-term) | 1% vs. adult. Reaching the adult level at 3 months of age | (6,7,9,10) |
Intestinal microbiota | Reduced motility, absence of the |
Almost absent. Reaching the adult activity at 6–12 months of age | (11-13) |
Serum albumin | <2.5 g/dL | <3 g/dL (vs. adult level of 4.2–4.6 g/dL) | (14) |
Bilirubin-albumin (B/A) binding | Larger inter-individual variability | Lower {(3–5) ×107 M−1} than adult albumin (6.7×106–108 M−1) | (15-17) |
Blood-CNS surfaces | |||
BBB | MVs sprouting starts at 8 Wg. Claudin expression similar to adult-one since 18 Wg. BBB unit establishment since 20 Wg | Fully established, but the still forming basal-lamina and astrocytic end-feet surrounding the MVs, might make them more fragile to |
(18-20) |
MVs density. Higher in the gray matter (about 111±30 MVs/mm2) vs. white matter (about 50±20 MVs/mm2) up to 32 Gw | MVs density. Gray matter: about 250±90 vs. white matter: about 90±22 MVs/mm2 at 40 Gw. Still |
||
CPs (forming the BCSFB) | Already present at 7 Wg, but still |
Claudin 2 more expressed postnatally | (21,22) |
ABCC1 [marker of the basolateral side (blood facing) of the choroid plexus (CPs)] | Already strongly expressed in CPs and ependyma since 22–26 Wg. Not detectable at the BBB (at any developmental age) | CPs: all ABC family C transporters are well expressed and active at birth | (23) |
Detectable in pyramidal and Purkinje cells of the cerebellum at 26 Wg, increasing up to 30 Wg | |||
ABCC2 (liver) | No data | 0.5% vs. adult. At 12 months, still only 1% vs. adult | (8) |
ABCC3 (liver) | Protein expression is fourfold lower in the fetus (16.4–37.9 weeks) than in infants and adults | Higher in infants and adults | (24) |
ABCB1 [marker of the endothelial cells forming the BBB, expressed at the apical side (blood facing) of the micro vessels (MVs)] | BBB. 22–26 Wg: detectable in 33–50% of samples of the brainstem, hindbrain ad thalamus. From 27 to 33 Wg, it became detectable everywhere | BBB. Detectable in MVs of all regions of the brain, but its expression is still increasing from birth to adult age | (23,25-28) |
Neurons. 22–26 Wg. Detectable in |
Neurons. 30 Wg: detectable in pyramidal neurons and h-Purkinje cells of the cerebellum (100% of the samples) | ||
ABCG2 (potential UCB |
Liver: 1.5 folds higher in fetus |
Liver: lower in infants and adults | (24-28) |
BBB: since 22 Wg detectable | No changes in expression both at the BBB and CPs (and until adult age). Possibly, its expression in CPs relies on the MVs inside the stroma | ||
CPs: no/barely detectable | |||
Detoxifying enzymes |
Detoxifying enzymes activity: all higher in CPs than in other CNS structures | Mainly expressed in CPs (vs. brain parenchyma or BBB). Higher postnatal activity (more than in adult age) | (28-34) |
SULT1A1 detectable since 15–20 Gw | NB: Gsts (rat samples) still high at birth in the LV CP, decreasing with development in the 4V CP, with the exception of GSTα (ligandin) that is lower at birth than in adult life | ||
NB: Gsts (rat samples) high since P2—representing approximately human 23–32 Wg) |
ABCC1: also known as multidrug protein 1 (MRP1); ABCC2: MRP2; ABCC3: MPR3; ABCB1: also known as P glycoprotein (PGP) or multidrug resistance protein 1 (MDR1); ABCG2: breast cancer resistance protein (BCRP). Wg, weeks of gestation; OATP, organic anion transporters; UGT, uridine 5' diphospho-glucuronosyltransferase; BBB, blood brain barrier; MVs, micro-vessels; CPs, choroid plexuses, forming the BCSFB, blood cerebro-spinal fluid barrier; Wg, weeks of gestation; ABC, ATP binding cassette transporters; CYP, cytochrome P450 oxygenase; GSTs, glutathione-S-transferase; GPs, glutathione peroxidase; SULT, sulfotransferase; UGT, uridine glucuronosyl-transferase; P, post-natal age in days; LV/4V CP, lateral and forth ventricle choroid plexuses.
The high hydrophobic nature of Bf makes it able to diffuse across the cellular bilayer and entering the cells, if not bound to serum albumin. Based on that, in neonates (especially in pre-terms), which have a lower serum albumin level than the adults (see later on in the review, as well as Table 1), serum albumin has to be considered as an additional determinant controlling the Bf entrance into the brain (37-42). The importance of serum albumin is well demonstrated also by animal models, where the brain damage in hyperbilirubinemic Gunn rats (43,44) crossbred with an-albuminemic rats occurs at much lower (¼) blood bilirubin level than in the (normo-albuminemic) Gunn strain (45). In addition, the affinity of neonatal albumin for bilirubin is lower than that of adult albumin (15,36), and largely variable between individuals (39,46-49).
At present and in the clinical practice, TSB is the value on which the indication of phototherapy (PT) is decided to prevent neurological damage. Nevertheless, due to the large and partly unpredictable variables able to interfere with the bilirubin-albumin binding (see later on in the text) and altering Bf, the recent advances making easier the quantification of Bf also in clinic (50) offer the opportunity of positively impact on the management of the condition.
Neonatal hyperbilirubinemia may lead to two opposite conditions: (I) the so-called physiological neonatal hyperbilirubinemia, un-risky and self-resolving in 1 to 2 weeks (36,51), not needing medical interventions, and (II) the severe neonatal hyperbilirubinemia, potentially leading to conditions ranging from mild-temporary deficits, to permanent neurological alterations, recapped under the definitions of bilirubin induced neurological dysfunction (BIND) and kernicterus spectrum disorder (KSD) (52-54).
From the “kern-icterus” to the KSD
For a long time, the term kernicterus has been used to identify the most dramatic consequences of bilirubin neurotoxicity in the course of severe neonatal hyperbilirubinemia. This term was coined by Schmorl in late 1903 to describe a specific pattern of yellow brain coloration (kern=core, nuclei; icterus = yellow) restricted to specific CNS structures in neonates who died with severe jaundice. Affected regions included the basal ganglia, the hippocampus, the central part of the cerebellum, and the wall of the third and fourth ventricle [reviewed in (51)].
In 1966, Diamond demonstrated that the portion of serum UCB able to enter the CNS is limited to Bf, with albumin (MW: 66 KDa), physically retaining the pigment into the vascular lumen (55). Conditions inducing an opening of the blood-brain interfaces (BBI) (for example hypoxia, hypercarbia, hyperosmolarity) will increase UCB brain content and alter the dynamics of clearance of the pigment from the brain (36,56-58). The same is true when blood UCB is displaced from serum albumin. In this respect, a long and ever-increasing list of displacing molecules exists, including typical drugs and nutritional approaches used especially in pre-term neonatal care (59-66). Altogether this data indicates that Bf crosses rapidly the BBI in either direction.
Due to the good agreement with the main clinical symptoms, the MR/proton MR spectroscopy findings (67-71), and some extreme experimental model where UCB entry into the brain was acutely increased by the use of displacing agents (72-76), the concept that brain damage was due to the accumulation—the so-called “deposition”—of bilirubin in specific CNS structures—the “kern-icterus”—became a dogma affecting the research of the field for decades.
Extensive work has been done searching the reasons for the selective UCB accumulation in specific brain areas. Differences in (in and out) transport (23,77-79), binding (78,80), metabolism (81), ability to oxidize, thus decrease, UCB level (74,82), as well as different circulatory rates among CNS regions, have been hypothesized, ever without replicating the “kern-icterus”.
In the meantime, bilirubin was discovered preferentially binding to myelin (41,83), alter the cellular membrane permeability and functions (84,85), inducing a bioenergetics crisis, apoptosis and necrosis, inflammation, redox and calcium imbalance, glutamate neurotoxicity, and synaptic excitability and transmission (82,85-95). It also alters the cell cycle (96), and acts on several cellular signaling pathways [reviewed in (29,71,97-99)].
Only in 2000, two reviews by TW Hansen (51,100) started criticizing the dogma. Hansen carried out a careful rereading of the history, reporting that, among the infants that died with jaundice, only marginal cases (less than 5%) presented the “kern-icterus”, and that in the majority of the autopsies the brain was diffusely yellow. Thus, bilirubin accumulation in specific brain areas is not required for developing neurological damage, although bilirubin toxicity affects specific structures of the CNS, while others areas are insensitive to bilirubin, despite the equal bilirubin level. This observation is well supported by recent in vitro (101), ex vivo (102) and in vivo animal studies (74,103).
One of the major alternative theories calls into play brain development, suggesting that the most affected regions of the brain are those in which key developmental processes are ongoing at the time of bilirubin challenging (104). In line with this conclusion are several experimental data.
In vivo (animal models), PT given to jaundiced animals at specific post-natal ages [P7 +/− 3 in Gunn rats (105)], fully protects from cerebellar hypoplasia. Supportive is the fact that the areas undergoing differentiation at the moment when bilirubin plasma concentration peaks show a higher bilirubin induced damage (73,105,106).
Recent ex vivo findings, obtained using organotypic brain cultures (OBCs) from rat pups at different post-natal ages exposed to UCB, demonstrated a developmental sensitivity to bilirubin toxicity (together with the regional sensitivity: hippocampus ˃ inferior colliculi = cerebral cortex), with the OBCs from an 8-day-old rat brain showing the maximal damage (102).
It is well known that neurons are more sensitive to bilirubin toxicity than astrocytes (29,107), with less differentiated neurons [based on the day in vitro (DIV) (108,109)] showing a higher degree of mitochondrial damage, oxidative and energetic crisis (101,110), altered neurogenesis and synaptogenesis, in addition to increased mortality (111,112). Notably, ABCC1/MRP1 expression (ATP binding cassette C1/multidrug resistance protein 1, a UCB transporter) increases with the neuronal maturation in vitro, and ABCC1 inhibition (by MK571), enhances UCB cellular entry (113). This suggests a role of this transporter in maintaining intracellular bilirubin below damaging concentration. Despite that, ABCC1 looks not to be sufficient to face the bilirubin challenge during severe neonatal hyperbilirubinemia in vivo (78,114), possibly due to its low expression in the brain parenchyma, moreover limited to few selected cellular populations (23,115).
An alternative explanation to the increased sensitivity in less mature cells/brain area has been hypothesized to be an undeveloped cellular defense [reviewed in (29)].
The oligodendrocytes are the second neuronal population largely sensitive to bilirubin toxicity. In primary culture of oligodendrocytes, UCB can interfere with maturation and differentiation, and then with the myelination process (29,103,116). Importantly, while neurogenesis in humans is a pre-natal event (possibly relevant in pre-term newborns), oligodendrogenesis is still present after birth in humans, with myelination occurring up to 10 years after birth (117). In agreement, altered myelination has been reported both in animal models (103,118) and at autopsy of infants died with kernicterus (114,119).
More recently bilirubin has been demonstrated to alter brain maturation by acting on the epigenetic of CNS development, including myelination, reinforcing the idea that bilirubin and CNS development are strictly interconnected (120). This hypothesis might be particularly important nowadays when the improvement of medical care allows more and more pre-term infants to survive. Thus, emerging is the need for a better understanding of the impact of CNS maturity (and immaturity) on the disease, as well as for a focus on bilirubin brain entry in pre- and full-term neonates.
Severe neonatal hyperbilirubinemia: full-term and pre-terms as two distinct populations
Hyperbilirubinemia in neonates is a benign condition in the majority of cases. However, an uncontrolled and rapidly increased blood bilirubin level can lead to neurotoxicity with even deadly consequences. Frequently considered an event of the past (121), the mortality of neonatal jaundice in the early neonatal period (0–6 days) still accounts for 1,309.3 deaths per 100,000 subjects worldwide (122). The burden is highest in the low-middle income countries (LMIC), especially in Sub-Saharan Africa and South Asia, where neonatal hyperbilirubinemia is the 7th and 8th leading cause of mortality, respectively. In Western Europe and North America, neonatal hyperbilirubinemia accounts for the 9th and 13th leading cause of mortality, respectively (122). Severe hyperbilirubinemia has been recognized as the cause of bilirubin-induced neurotoxicity which can manifest as acute brain encephalopathy (ABE), KSD, and death (123,124).
The risk threshold: TSB is not enough
Several clinical differences exist among full [≥37 weeks gestational age (Wg)] and pre-term neonates (<37 Wg) (see Table 2) exposed to hyperbilirubinemia. Neonatal hyperbilirubinemia affects 60% of full-term and 80% of pre-term neonates (10,125,126), with pre-term infants having a higher risk of severe jaundice with or without bilirubin-induced neurotoxicity than do full-term infants (148-150). The increased percentage of hyperbilirubinemia in pre-terms is mainly due to the enhanced (with respect to full term neonates) fragility of the red blood cell (bilirubin production), the increased liver (transport and conjugation) and intestinal immaturity, and postponed enteral feeding (7,10,148) (see Table 1).
Table 2
Variable | Pre-term | Full term | Ref. |
---|---|---|---|
Gestational age | <37 weeks | ≥37 weeks | |
Prevalence of hyperbilirubinemia | 80% | 60% | (10,125,126) |
Incidence in infants with severe hyperbilirubinemia (TSB >20 mg/dL)* | |||
ABE* | 66.6% (n=20/30) | 87.6% (n=80/89) | (127) |
KSD* | 93% (n=27/29) | 86.4% (n=83/96) | |
Death* | 3.45% (n=1/29) | 5.2% (n=5/96) | |
Clinical manifestation | |||
ABE | More subtle, recurrent apnea and desaturation | Classic ABE signs: initial phase: stupor (lethargy), hypotonia, and poor sucking; Advanced phase: hypertonia, arching, retrocollis, opisthotonos, fever, high-pitched cry, apnea, inability to feed | (52,128-131) |
KSD | Equally the same with term neonates with auditory neuropathy spectrum disorder predominant | Athetoid cerebral palsy (uncontrollable movement of the face, body, arms and legs), hearing loss, failure of upward gaze and dental enamel dysplasia | |
Incidence of apnea associated with ABE | ≥35 to <37 weeks: n=14/28; 50% | ≥37 weeks: n=27/80; 33.8% | (132) |
% of abnormal ABR in hyperbilirubinemic infants | 25% (n=9/36) | 14% (n=11/80) | (60) |
Parameter for diagnosis | TSB, plus birth weight, serum albumin, and gestational age | TSB alone | (128,133-139) |
Mean TSB | 23–34 weeks: 5.4±1.4 mg/dL; |
≥37 weeks: 7.9±1.6 mg/dL; |
(59,60,140) |
Mean Bf | 23–34 weeks: 13.1±8.4 nM; ≥28–34 weeks: 17.8±9.7 nM | ||
Peak Bf in infants with abnormal ABR | ˂28 weeks: n=9/36; 19.0±15.9 nM | – | (39) |
≥28–34 weeks: n=11/80; 21.9±21.1 nM | |||
28–32 weeks: n=25/45; 10.5±3.4 nM | – | (141) | |
24–35 weeks: n=6/81; 30.1±22.4 nM | – | (142) | |
≥34 weeks n=24/100; 57.6±49.9 nM | (143) | ||
Peak of Bf in infants with central apnea | 27–33 weeks: n=7/50; 39.3±44.4 ìg/dL | (144) | |
Treatments | |||
Phototherapy | Prophylactic PT | Perform as conservative mode | (59,60,145) |
Possibly inefficient in reducing Bf and largely affected by displacing compounds | |||
% of TSB/Bf decrease after PT | 43%/null | 29%/19% | (59) |
Exchange transfusion (ET) | Based on TSB level threshold and gestational age, and the presence of ABE sign (less obvious) | Based on TSB level and the presence of ABE sign (most obvious) | (146) |
Deaths within 7 days following ET | 5% [37/704] | 1% [5/457] | (147) |
*, the incidence of ABE, KSD, and death are among the infants with severe hyperbilirubinemia (TSB >20 mg/dL) only. Neither the healthy infants nor moderate-mild hyperbilirubinemia were included. ABE, acute brain encephalopathy; KSD, kernicterus spectrum disorder; TSB, total serum bilirubin; Bf, free bilirubin; PT, phototherapy.
While the TSB peak plotted as a function of the hours after birth is sufficient to estimate the risk of neurological sequelae in term infants (133,134), additional factors are required in pre-term babies (see Table 2). In this population, common is the so-called “low bilirubin kernicterus”, in which the neurological damage (symptoms and/or neuroimaging findings of brain damage) may be present even with peak TSB under the “safe level” (128,135-138). In pre-term babies, usually, bilirubin induced brain damage is associated with extremely low birth weight (ELBW) and/or strikingly low serum albumin level (1.4 to 2.1 g/dL) (135,137,138). Indeed, at equal TSB, the risk of developing neurological sequela increases as the gestational age decreases, as reported for example by Bhutani and Wong (e.g., 10% below: 30 Wg, 5.5% below 31–32 Wg, and 1.2% below 33/34 Wg). Thus, rather than a specific threshold, in pre-term infants a range of TSB levels is more likely to be associated with the onset of neurotoxicity (133). Both body weight and serum albumin level might be considered additional (possibly personalized) indicators of the developmental maturity of the infant, in addition to the gestational age, all affecting the Bf. In agreement with it, the presence of neurological dysfunctions or even death in ELBW pre-term infants have been associated with a high level of free/unbound bilirubin (139,151), suggesting Bf as a more sensitive predictor in respect to TSB (141,152). Bf has also been found as a good predictor for auditory dysfunctions in neonates with severe jaundice (142,143,153,154), as well as for the risk of apnea in pre-term infants (144,155). The bilirubin neurotoxicity affecting auditory system including brainstem auditory nuclei, vestibular nuclei, and auditory nerve, has been reproduced in both and animal model of kernicterus (Gunn rat) (156,157). Nevertheless, the interplay between body weight, serum albumin level, Bf and the immaturity of the brain, one of the reasons for the increased susceptibility to neuronal damage in the pre-term population even at lower bilirubin levels (135), still has to be fully unravelled. Reliable experimental models are required.
The clinical manifestations
Full-term infants
In mature infants, lethargy, hypotonia, and poor sucking are the early non-specific sign of acute bilirubin encephalopathy (ABE) (Table 2), with hypertonia (retrocollis and opisthotonos), fever and high-pitched cry, apnea, and inability to feed representing the signs of advanced stage of ABE.
The classical sign of the chronic and permanent clinical sequelae of bilirubin toxicity in term population includes motor symptoms, hearing loss due to auditory neuropathy spectrum disorder (ANSD) with or without hearing loss, visual impairment (visuo-oculomotor, usually manifests as paralysis of upward gaze, and visuo-cortical dysfunction), and dental enamel dysplasia (54,129,130). Those symptoms present a large variability among individuals, recently recapped by the term KSD (52). In addition, the neurodevelopmental sequelae, later described as spectrum of developmental disorder, have been considered as part of bilirubin-induce neurotoxicity disorders. Bilirubin-induced cognitive delay, attention deficit hyperactivity disorder (ADHD), autism spectrum disorder (ASD), specific learning disorder and language disorder are neurodevelopmental disorders (NDDs) that have been associated with bilirubin neurotoxicity (53,54).
The stage of brain development (the main biological mechanisms: e.g., division, differentiation, migration, myelination, etc.) at the time of bilirubin insult has been hypothesized as one of the possible explanations for this variability, as discussed above. However, hyperbilirubinemia in un-treated full-term neonates may persist up to 1 or 2 weeks after birth, and, this might be too short a time to represent different stages of the CNS development. An alternative explanation may reside in individual genetic susceptibility, explaining also why kernicterus may develop under the TSB risk threshold, or, vice versa, do not develop despite franc toxic TSB levels (158).
Pre-term infants
Pre-term infants less frequently exhibit the conventional bilirubin neurotoxicity signs (Table 2), likely due to incomplete maturation of neuronal circuit and organization. For this reason, pre-term infants are more at risk of late-diagnosis or even stay undiagnosed, which lead them to suffer from “silent morbidity and mortality” (130,159). Meanwhile, the auditory predominant sequelae are more common in pre-term neonates (128,130,131). Pre-term infants with abnormal auditory brain evokes responses (ABR, the common clinical test performing among pre-term infant to evaluate brainstem function related to auditory neural pathway), also present a more concurrent apneic events (155), possibly because both these conditions share the same neuronal location targeted by bilirubin. Moreover, increasing are the evidences reporting that hyperbilirubinemia in premature infants is followed by a higher number of apnea events (135,144,160).
Concerning the chronic and permanent clinical sequelae of bilirubin toxicity, pre-terms share the same burden as that of the full-term neonates (129,130). This may suggest that, under chronic toxic stimuli, inducing the most severe molecular perturbations and cell death (71,104,161), the developmental stage of brain development is irrelevant.
Various clinical studies tried to link the higher prevalence of NDDs (global cognitive delay, ADHD, specific learning disorder, ASD) in pre-term infants with hyperbilirubinemia but none showed a significant association (53). It must be underlined that most of them used TSB or UCB, but not Bf.
Notably, the occurrence of athetoid cerebral palsy in more than 6 months old infants have been noticed among pre-term infants with a history of moderate hyperbilirubinemia (7–17.4 mg/dL) and despite PT during the neonatal period (128,136). A potential explanation might come from the recent and disturbing finding that PT may be ineffective in reducing Bf in pre-term infants, despite a significant decrease of the TSB (49,59,65). This dichotomy remains still unexplained. Certainly, pre-term infants often require in addition to PT drugs and nutritional approaches, and, among them, several can interfere with the bilirubin to albumin binding, increasing Bf [as previously discussed (48,65,66)].
Therapeutical approaches
Phototherapy
PT is the standard treatment for neonatal hyperbilirubinemia to convert bilirubin into water-soluble photoisomers that can be excreted through bile and urine. Effective PT has progressively decreased the need for exchange transfusion (ET) in pre-term infants (145). Recently, double-PT, which use two light sources, has been demonstrated more effective for reducing TSB level compare to single PT among pre-term infants (162).
In addition to the emerging inefficacy of PT in pre-term babies (see above), PT is not a harmless treatment and overtreatment should be reevaluated in small pre-term infants (Table 2). Side effects can include retinal damage, burns, disturbed circadian rhythm, conjunctivitis, rashes, dehydration, hyper- and hypothermia, loose stools, melanotic nevus, bronze baby syndrome, and electrolyte disturbances (163).
In a large randomized control trial aggressive (prophylactic) PT was compared with conservative PT in ELBW (≤1,000 g) infants. Aggressive PT was provided at a TSB value of 5 mg/dL or higher in the first week and 7 mg/dL or higher in the second week. Meanwhile, conservative PT was provided at a bilirubin value of 8 mg/dL or higher for 501–750 g infants and 10 mg/dL or higher for 751–1,000 g infants. This study showed that aggressive PT in ELBW infants reduced neurodevelopmental impairment and hearing loss among surviving infants versus those receiving conservative PT. However, those results are offset by the post hoc analysis reporting a 99% probability of increased deaths among infants under 750 g birth weight with aggressive PT (164). These results suggest that moderate bilirubin levels may have clinically important oxidant benefits (165). Low concentration of bilirubin scavenges reactive oxygen species (ROS), reduces oxidant-induced cellular injury and attenuates oxidant stress. Since the physiologic jaundice has to be accepted as a protective mechanism for the newborn infant against ROS in the first days of life (163), aggressive, prophylactic PT looks to be counterproductive.
Indeed, the thin, translucent skin of ELBW infants and their high rate of serious illness and immature defense mechanisms may make them particularly vulnerable to the potential or documented adverse effects of PT, including photo-oxidative injury, lipid peroxidation, DNA damage, reduced mesenteric and cerebral blood flow, and hemolysis (163,166). Interestingly, a recent study reported that the cycled (intermittent) PT can reduce the mortality associated with continuous PT among the pre-term infant (167).
In any case, PT has significantly decreased the overall incidence of bilirubin neurotoxicity in most developed countries. Nevertheless, bilirubin neurotoxicity with lifelong neurological sequelae still occurs, and is a major problem in many areas of the world, especially in low- and middle-income countries (168,169). The access to health facilities, availability of PT, the possibility to measure TSB at the side of the newborn, and the variation in PT practices (such as the irradiance distances between infants and the light sources) have contributed to sub-optimal result of this therapeutical approach (168,170).
Exchange transfusion
ET is the standard therapy for severe hyperbilirubinemia with ABE (146). ET will be performed if the hyperbilirubinemia exceeds the specific level defined in clinical guidelines and exposes infants tot the risk of bilirubin neurotoxicity (134). The incidence of severe hyperbilirubinemia adjusted according to the American Academy of Pediatrics thresholds for ET is low, involving ~1.2 per 1,000 live births (171). The screening of hyperbilirubinemia and its underlying condition [e.g., rhesus and ABO isoimmunization, glucose 6 phosphate deficiency (G6PD)], the treatment of pregnant women who are Rh-negative with Rh-factor therapy, and the increased use of PT, have drastically reduced the number of ET performed (147). Nevertheless, premature infants have a tenfold increased risk of eventual bilirubin level meeting or exceeding thresholds for ET compared with term neonates (171).
Furthermore, most studies suggest that sick pre-term infants experience a wide range of complications from ET more frequently than term infants (145,147,172,173). In a large cohort study of >1,200 pre-term and term infants who received ET for hyperbilirubinemia, pre-term infants especially those ≤29 weeks of gestational age, have greater odds of death following ET compared to term infants (147).
The novel treatment strategies
Despite PT and ET have been widely accepted as standard treatments for severe hyperbilirubinemia to prevent ABE, the limitation of both therapies calls for new neuroprotective treatment.
Minocycline, the anti-inflammatory and antimicrobial drug, has been effectively demonstrated to prevent kernicterus in animal models of hyperbilirubinemia (174-176). However, the use of minocycline in neonates is prevented due to its inevitable side effects including tooth discoloration, increased skin hypersensitivity to light, skin and nail hyperpigmentation, and skin rash (146).
Other drugs under study for treating hyperbilirubinemia is tin-mesoporphyrin (SnMP), the potent competitive inhibitors of heme oxygenase, the key rate-limiting enzyme in the catabolism of heme to bilirubin (177). Reddy et al. have reported a very low birth weight infant with severe hyperbilirubinemia which, while awaiting an ET, underwent a SnMP single-dose treatment. After 10 hours of SnMP administration, TSB was gradually reduced (by 13%) and this eliminated the need for ET. No adverse effects were reported (178). A clinical trial of SnMP in 213 newborns has shown the early use of a single dose of SnMP decreased the duration of PT, reversed TSB trajectory (mean TSB declined by 18%), and reduced the severity of subsequent hyperbilirubinemia. However, data on long-term risk of BIND still lack in this study (179).
A possible alternative approach might be focused on counteracting directly into the brain the molecular mechanisms of damage triggered by bilirubin, irrespective of the TSB (102). A recently published in vivo work reported that curcumin was able to fully restore brain damage and behavioral abnormalities in the spontaneously hyperbilirubinemic Gunn rat by counteracting the main pathological mechanisms of CNS damage (118). This approach might be useful where PT/ET are not available or efficient in (otherwise healthy) full-term hyperbilirubinemic newborns.
From the serum to the brain: the BBI in brain development and KSD
To enter the brain, Bf has to cross the BBI, located at the endothelial walls of the brain vasculature [blood-brain barrier (BBB)], and at the epithelial layer of the choroid plexuses (CPs), the latter giving access to the cerebrospinal fluid (CSF) (blood-CSF barrier, BCSFB) (Figures 1,2). The barrier cells are sealed by tight junctions, and harbor multi-specific efflux transporters and detoxifying enzymes that altogether prevent the diffusion of unwanted compounds into the brain or else increase the clearance of potentially harmful metabolites from the brain (181). They also fulfill an important brain delivery function for energy substrates, micronutrients and hormones, and contribute to the specific immune privilege of the brain (21,182). Contrary to the received idea of brain barriers lacking maturity during perinatal development, both interfaces display a barrier phenotype very early during fetal life, and are functionally efficient to supply the nervous system with nutrients and biologically active molecules that match the specific needs of the developing brain.
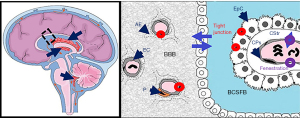
Development and differentiation of brain barriers
Brain barriers during pre- and postnatal brain development
Vascularization of the brain is an early event. It starts on embryonic day 9.5 in mice, when endothelial cells from the perineural vascular plexus invade the neuroepithelium, a process regulated by the canonical Wnt/β-catenin signaling. This pathway orchestrates the development of the vascular network, controls the secretion of extracellular matrix proteins, and initiates the induction in endothelial cells of their brain-specific properties such as the expression of glucose transporter 1 (GLUT-1) allowing glucose to fuel the brain (183). The endothelium of the newly formed vessels displays functional tight junctions characterized by the focal localization of tight junctions-associated proteins such as occludin and claudin 5, and reduced pinocytosis activities, both typical of the BBB phenotype. Pericytes play an important part in inducing these latter properties (184,185). Astrocytes that mature essentially postnatally in rodents, play a role in the maintenance of the BBB phenotype later on (Figure 2). In the postnatal brain, the surface of exchange across the BBB is more limited than in the adult because the neurovascular network develops and complexifies gradually throughout development, in two waves of angiogenesis, one prenatal, the other postnatal (180).
Morphological and immunohistological studies in humans indicate a pattern a vascularization and BBB development similar to that observed in rodents, although with a different timeframe. Vascularization of the telencephalon starts around week 8 of gestation (Wg) (Table 1), characterized by the co-migration of endothelial sprouts and intimately associated pericytes (18). The tight junction-associated proteins occludin and claudin 5 are expressed in endothelial cells on week 12 Wg, and by week 18 Wg these proteins display an intercellular localization pattern similar to that of the adult. The blood vessel density and the percentage of blood vessel area are largest in the germinative matrix exposed to nascent CSF followed by gray matter and then the white matter in all of the gestational age between 16 and 40 weeks (19). In humans, astrocyte end-feet start to escheat endothelial cells before birth around week 20 Wg depending on the brain region (20). This is consistent with the mainly postnatal development of end-feet in rodents, as rat neonates are considered to be equivalent to human fetuses of that stage in terms of cortical development.
Despite the early appearance of a typical morphologic BBB phenotype characterizing the developing brain vessels, the more limited thickness of the basal membrane and the gradual covering by astrocytic end-feet suggest that the BBB maybe more fragile and prone to disruption during the peri and early postnatal period than in the adult.
The CPs start developing from the dorsal part of the neural tube even before the vasculature becomes significant in fetal life, at the seventh week of gestation in humans (Table 1).The concurrent formation of the choroidal epithelium and vascular conjunctive stroma (Figure 2) is complex, interrelated, and involves bone morphogenic proteins such as Gdf7 gene product, the homeobox protein Otx2, and sonic hedgehog signaling. The CPs mature early to present an “adult” phenotype at birth (Table 1). They keep extending after birth however to match brain growth, as a result of an active mitogenic epithelial area at the root of the CPs. A detailed description of CP development and differentiation is described in (21,22). The choroidal epithelium displays a tight phenotype as soon as it invaginate from the neural tube, and the signaling mechanism associated with this barrier phenotype has yet to be identified. The CPs are also an important transport/secretion site of nutrients and hormones delivery to the developing brain (21,186,187). The CPs epithelial tight junctions are made of a large number of different proteins, including the transmembrane proteins occludin, claudin1, claudin 2, and claudin 3. The relative proportion of these proteins however changes with development, with claudin 2 being more expressed postnatally and in adults, and claudin 3 being more expressed prenatally, possibly in connection with the selective choroidal function linked to CSF secretion which increases after birth (30).
Efflux transporters and enzymes that participate in the neuroprotective functions of brain barriers present a variable degree of expression and function at birth, which also differ between the cerebral vasculature and the CPs (Table 1). The BCSF barrier achieves an adult phenotype earlier than the BBB. This may be linked to the sensitivity of selected efflux transporters to the signals originating from the astrocytic endfeet, the later maturing mainly during the peri- and the postnatal period (188). For instance, at the BBB, the expression of several efflux transporters such as ABCB1 (PGP; MDR1), ABCG2 (BCRP), ABCC4 (MRP4) but not of others (SLC22A8/OAT3) increases from birth to adult stage. This is especially patent for ABCB1 which is 5 times lower in the early postnatal stages than in adults (25-28). In CPs, the main efflux transporters are ABCC proteins (MRPs), and they are well expressed and active at birth. Glutathione-S-transferases and glutathione-peroxidases, the sulfotransferase SULT1A1 and at least in rodents, UDP-glucuronosyl transferases are detoxification enzymes mainly found in the CPs, that keep the concentration of various toxicants low in the brain. Most of them are especially active postnatally (31-34).
Permeability of BBB to bilirubin
As the paracellular pathway across brain barriers is sealed by tight junctions, and non-specific pinocytosis is very low at brain barriers, protein-bound UCB has no access to the brain, except for a small fraction that could access the CSF across specific protein transporting cells from the choroidal epithelium during development (189).
In contrast, tight junctions are not a hindrance to the brain penetration of lipophilic Bf, which occurs through a transmembrane diffusional pathway (see Figure 1). Several mechanisms may however limit this diffusion of Bf in the postnatal brain.
ABCC1, expressed mainly at the BCSFB, and to some extent ABCB1, expressed mainly at the BBB, recognize UCB as a substrate. These efflux proteins will pump part of UCB back into the blood. The process however is not expected to be very efficient in neonates as the expression of ABCB1, whose affinity for UCB is not high, is still low in brain capillaries at that developmental stage (see Table 1). UCB is also metabolized by UGT1A1 (see Figure 1). While choroidal UGT1A-dependent enzymatic activities are high during postnatal development (Table 1), whether the specific UGT1A1 isoform is active is not known. UCB can be oxidized by cytochrome P-450-dependent monooxygenases, such as CYP1A1/1A2 (Figure 1). This is however unlikely to constitute an important hindrance to the passage of UCB across the brain barriers, as CYP are not major detoxification enzymes at brain barriers [reviewed in (31,190)]. UCB transfer to the brain can also be slowed down through binding to glutathione-S-transferase (GST) subunits alpha, but the expression of these specific isoforms raises only postnatally in brain barriers, hence this mechanism is unlikely to be significant in neonates.
Altogether the evidence points to transport and metabolic mechanisms at undamaged brain barriers that may prevent or reduce the diffusion into the brain of unbound UCB circulating at a physiologically very low level in the plasma, but that should be overrun if unbound UCB reaches higher pathological levels [reviewed in (31)]. The regional differences that have been observed in brain sensitivity to UCB appear not linked to regional differences in barrier permeability to UCB. Whether localized differences in interstitial fluid movements, and CSF flow, impact the cerebral bio-disposition of UCB, and influence this regional susceptibility remains to be investigated.
Effect of bilirubin on BBB functions
Brain interfaces are the first cerebral cells exposed to the high concentration of UCB circulating in the plasma during pathological neonatal jaundice. They may therefore be a primary target for UCB toxicity, as an alteration in the development of the neurovascular network and CPs, and of their associated barrier phenotype, would impact brain maturation.
So far, few investigations have been performed on this matter, and most are in vitro studies using cultured brain endothelial and epithelial cells. The integrity of the endothelial monolayers was found sensitive to UCB, while that of the choroidal epithelial monolayers was not (115,191).
Because BBB models do not yet recapitulate all features of BBI in vivo, more information from in vivo studies needs to be collected before a conclusion can be drawn on the effect of free UCB on brain barriers integrity. A case report of a pre-term neonate with severe kernicterus indicates that signs of neurovascular network alteration was observed on autopsied brain tissue, possibly linked to an increase in vascular endothelial growth factor (VEGF) signaling (192). Given the comorbidities associated, whether UCB alone was responsible for these alterations remains to be understood. One study performed in Gunn rats, an animal model of jaundice characterized by a rapid postnatal increase in serum UCB evidenced a decrease in ABCC1 protein levels in CPs. This finding could be reproduced in vitro on choroidal epithelial cells chronically exposed to UCB, suggesting that ABCC1 downregulation results from a direct effect of UCB on the BCSFB (115). In a model of bile duct ligation, a decrease in ABCG2 was observed at the BBB, with evidence that UCB mediates the effect, and without apparent impairment of the integrity of the barrier (193).
These in vivo data suggest that UCB induces functional changes at brain barriers, rather than overt impairment of their integrity.
Conclusions
In the last decades, a lot of bench-based work has been performed to unravel bilirubin-induced neurotoxicity. Some “dogma” fell and new knowledge raised new hypotheses that need to be experimentally and clinically explored by the “yellow researchers”. The cooperative, international, and multidisciplinary expertise will be the key to success.
Acknowledgments
Funding: This work was supported by ANR-10-IBHU-003 Cesame grant, to JFGE, an internal grant from Fondazione Italiana Fegato - ONLUS to SJ and SG, a grant from Progetti Internazionali 2020 (DGR 2195 dd 20/12/2019), and a grant from LPDP to SJ.
Footnote
Provenance and Peer Review: This article was commissioned by the Guest Editors (David K. Stevenson and Ronald J. Wong) for the series “Neonatal Jaundice” published in Pediatric Medicine. The article has undergone external peer review.
Conflicts of Interest: All authors have completed the ICMJE uniform disclosure form (available at http://dx.doi.org/10.21037/pm-21-5). The series “Neonatal Jaundice” was commissioned by the editorial office without any funding or sponsorship. The authors have no other conflicts of interest to declare.
Ethical Statement: The authors are accountable for all aspects of the work in ensuring that questions related to the accuracy or integrity of any part of the work are appropriately investigated and resolved.
Open Access Statement: This is an Open Access article distributed in accordance with the Creative Commons Attribution-NonCommercial-NoDerivs 4.0 International License (CC BY-NC-ND 4.0), which permits the non-commercial replication and distribution of the article with the strict proviso that no changes or edits are made and the original work is properly cited (including links to both the formal publication through the relevant DOI and the license). See: https://creativecommons.org/licenses/by-nc-nd/4.0/.
References
- Cui Y, König J, Leier I, et al. Hepatic uptake of bilirubin and its conjugates by the human organic anion transporter SLC21A6. J Biol Chem 2001;276:9626-30. [Crossref] [PubMed]
- Briz O, Romero MR, Martinez-Becerra P, et al. OATP8/1B3-mediated cotransport of bile acids and glutathione: an export pathway for organic anions from hepatocytes? J Biol Chem 2006;281:30326-35. [Crossref] [PubMed]
- Gazzin S, Masutti F, Vitek L, et al. The molecular basis of jaundice: An old symptom revisited. Liver Int 2017;37:1094-102. [Crossref] [PubMed]
- Ahlfors CE, Marshall GD, Wolcott DK, et al. Measurement of unbound bilirubin by the peroxidase test using Zone Fluidics. Clin Chim Acta 2006;365:78-85. [Crossref] [PubMed]
- Hahm JS, Ostrow JD, Mukerjee P, et al. Ionization and self-association of unconjugated bilirubin, determined by rapid solvent partition from chloroform, with further studies of bilirubin solubility. J Lipid Res 1992;33:1123-37. [Crossref] [PubMed]
- Rubaltelli FF. Bilirubin metabolism in the newborn. Biol Neonate 1993;63:133-8. [Crossref] [PubMed]
- Watchko JF, Maisels MJ. Jaundice in low birthweight infants: pathobiology and outcome. Arch Dis Child Fetal Neonatal Ed 2003;88:F455-8. [Crossref] [PubMed]
- Mooij MG, Schwarz UI, de Koning BAE, et al. Ontogeny of human hepatic and intestinal transporter gene expression during childhood: age matters. Drug Metab Dispos 2014;42:1268-74. [Crossref] [PubMed]
- Onishi S, Itoh S, Kawade N, et al. An accurate and sensitive analysis by high-pressure liquid chromatography of conjugated and unconjugated bilirubin IX-alpha in various biological fluids. Biochem J 1980;185:281-4. [Crossref] [PubMed]
- Olusanya BO, Kaplan M, Hansen TWR. Neonatal hyperbilirubinaemia: a global perspective. Lancet Child Adolesc Health 2018;2:610-20. [Crossref] [PubMed]
- Gritz EC, Bhandari V. The human neonatal gut microbiome: a brief review. Front Pediatr 2015;3:17. [PubMed]
- Morelli L. Postnatal Development of Intestinal Microflora as Influenced by Infant Nutrition. J Nutr 2008;138:1791S-1795S. [Crossref] [PubMed]
- Vítek L, Kotal P, Jirsa M, et al. Intestinal colonization leading to fecal urobilinoid excretion may play a role in the pathogenesis of neonatal jaundice. J Pediatr Gastroenterol Nutr 2000;30:294-8. [Crossref] [PubMed]
- Watchko JF, Spitzer AR, Clark RH. Prevalence of Hypoalbuminemia and Elevated Bilirubin/Albumin Ratios in a Large Cohort of Infants in the Neonatal Intensive Care Unit. J Pediatr 2017;188:280-286.e4. [Crossref] [PubMed]
- Roca L, Calligaris S, Wennberg RP, et al. Factors affecting the binding of bilirubin to serum albumins: validation and application of the peroxidase method. Pediatr Res 2006;60:724-8. [Crossref] [PubMed]
- Ahlfors CE, Wennberg RP, Ostrow JD, et al. Unbound (free) bilirubin: improving the paradigm for evaluating neonatal jaundice. Clin Chem 2009;55:1288-99. [Crossref] [PubMed]
- Cashore WJ, Oh W. Unbound bilirubin and kernicterus in low-birth-weight infants. Pediatrics 1982;69:481-5. [PubMed]
- Virgintino D, Girolamo F, Errede M, et al. An intimate interplay between precocious, migrating pericytes and endothelial cells governs human fetal brain angiogenesis. Angiogenesis 2007;10:35-45. [Crossref] [PubMed]
- Ballabh P, Braun A, Nedergaard M. Anatomic analysis of blood vessels in germinal matrix, cerebral cortex, and white matter in developing infants. Pediatr Res 2004;56:117-24. [Crossref] [PubMed]
- El Khoury A, Gruber SH, Mørk A, et al. Adult life behavioral consequences of early maternal separation are alleviated by escitalopram treatment in a rat model of depression. Prog Neuropsychopharmacol Biol Psychiatry 2006;30:535-40. [Crossref] [PubMed]
- Ghersi-Egea JF, Strazielle N, Catala M, et al. Molecular anatomy and functions of the choroidal blood-cerebrospinal fluid barrier in health and disease. Acta Neuropathol 2018;135:337-61. [Crossref] [PubMed]
- Strazielle N, Ghersi-Egea JF. Physiology of blood-brain interfaces in relation to brain disposition of small compounds and macromolecules. Mol Pharm 2013;10:1473-91. [Crossref] [PubMed]
- Daood M, Tsai C, Ahdab-Barmada M, et al. ABC transporter (P-gp/ABCB1, MRP1/ABCC1, BCRP/ABCG2) expression in the developing human CNS. Neuropediatrics 2008;39:211-8. [Crossref] [PubMed]
- Mooij MG, van de Steeg E, van Rosmalen J, et al. Proteomic Analysis of the Developmental Trajectory of Human Hepatic Membrane Transporter Proteins in the First Three Months of Life. Drug Metab Dispos 2016;44:1005-13. [Crossref] [PubMed]
- Baello S, Iqbal M, Gibb W, et al. Astrocyte-mediated regulation of multidrug resistance p-glycoprotein in fetal and neonatal brain endothelial cells: age-dependent effects. Physiol Rep 2016;4:e12853 [Crossref] [PubMed]
- Gazzin S, Strazielle N, Schmitt C, et al. Differential expression of the multidrug resistance-related proteins ABCb1 and ABCc1 between blood-brain interfaces. J Comp Neurol 2008;510:497-507. [Crossref] [PubMed]
- Omori K, Tachikawa M, Hirose S, et al. Developmental changes in transporter and receptor protein expression levels at the rat blood-brain barrier based on quantitative targeted absolute proteomics. Drug Metab Pharmacokinet 2020;35:117-23. [Crossref] [PubMed]
- Strazielle N, Ghersi-Egea JF. Efflux transporters in blood-brain interfaces of the developing brain. Front Neurosci 2015;9:21. [Crossref] [PubMed]
- Brites D. Bilirubin Injury to Neurons and Glial Cells: New Players, Novel Targets, and Newer Insights. Semin Perinatol 2011;35:114-20. [Crossref] [PubMed]
- Kratzer I, Vasiljevic A, Rey C, et al. Complexity and developmental changes in the expression pattern of claudins at the blood-CSF barrier. Histochem Cell Biol 2012;138:861-79. [Crossref] [PubMed]
- Ghersi-Egea JF, Gazzin S, Strazielle N. Blood-brain interfaces and bilirubin-induced neurological diseases. Curr Pharm Des 2009;15:2893-907. [Crossref] [PubMed]
- Kratzer I, Strazielle N, Saudrais E, et al. Glutathione Conjugation at the Blood-CSF Barrier Efficiently Prevents Exposure of the Developing Brain Fluid Environment to Blood-Borne Reactive Electrophilic Substances. J Neurosci 2018;38:3466-79. [Crossref] [PubMed]
- Richard K, Hume R, Kaptein E, et al. Sulfation of thyroid hormone and dopamine during human development: ontogeny of phenol sulfotransferases and arylsulfatase in liver, lung, and brain. J Clin Endocrinol Metab 2001;86:2734-42. [Crossref] [PubMed]
- Saudrais E, Strazielle N, Ghersi-Egea JF. Choroid plexus glutathione peroxidases are instrumental in protecting the brain fluid environment from hydroperoxides during postnatal development. Am J Physiol Cell Physiol 2018;315:C445-56. [Crossref] [PubMed]
- Daood MJ, Watchko JF. Calculated in vivo free bilirubin levels in the central nervous system of Gunn rat pups. Pediatr Res 2006;60:44-9. [Crossref] [PubMed]
- Gourley GR. Bilirubin metabolism and kernicterus. Adv Pediatr 1997;44:173-229. [PubMed]
- Ahlfors CE, Herbsman O. Unbound Bilirubin in a Term Newborn with Kernicterus. Pediatrics 2003;111:1110-2. [Crossref] [PubMed]
- Bhutani VK, Wong RJ, Stevenson DK. Hyperbilirubinemia in Preterm Neonates. Clin Perinatol 2016;43:215-32. [Crossref] [PubMed]
- Hegyi T, Kleinfeld A, Huber A, et al. Unbound bilirubin measurements by a novel probe in preterm infants. J Matern Fetal Neonatal Med. 2019;32:2721-6. [Crossref] [PubMed]
- Wennberg RP, Ahlfors CE, Bhutani VK, et al. Toward Understanding Kernicterus: A Challenge to Improve the Management of Jaundiced Newborns. Pediatrics 2006;117:474-85. [Crossref] [PubMed]
- Brodersen R. Binding of Bilirubin to Albumin and Tissues. In: Minkowski A, Monset-Couchard M. editors. Physiological And Biochemical Basis For Perinatal Medicine. 1981:144-52.
- Bratlid D. How bilirubin gets into the brain. Clin Perinatol 1990;17:449-65. [Crossref] [PubMed]
- Chowdhury JR, Kondapalli R, Chowdhury NR. Gunn rat: a model for inherited deficiency of bilirubin glucuronidation. Adv Vet Sci Comp Med 1993;37:149-73. [PubMed]
- Gunn CH. Hereditary acholuric jaundice in a New Mutant Strain of Rats. J Hered 1938;29:137-9. [Crossref]
- Takahashi M, Sugiyama K, Shumiya S, et al. Penetration of Bilirubin into the Brain in Albumin-Deficient and Jaundiced Rats (AJR) and Nagase Analbuminemic Rats (NAR). J Biochem 1984;96:1705-12. [Crossref] [PubMed]
- Cashore WJ. Free bilirubin concentrations and bilirubin-binding affinity in term and preterm infants. J Pediatr 1980;96:521-7. [Crossref] [PubMed]
- Wennberg RP, Ahlfors CE, Rasmussen LF. The pathochemistry of kernicterus. Early Hum Dev 1979;3:353-72. [Crossref] [PubMed]
- Amin SB. Bilirubin Binding Capacity in the Preterm Neonate. Clin Perinatol 2016;43:241-57. [Crossref] [PubMed]
- Amin SB, Wang H. Bilirubin Albumin Binding and Unbound Unconjugated Hyperbilirubinemia in Premature Infants. J Pediatr 2018;192:47-52. [Crossref] [PubMed]
- Gazzin S, Riordan SM. Commentary on the Don Ostrow Trieste Yellow Retreat 2019: a successful biennium, what next? Pediatr Res 2020;88:372-3. [Crossref] [PubMed]
- Hansen TW. Pioneers in the scientific study of neonatal jaundice and kernicterus. Pediatrics 2000;106:E15 [Crossref] [PubMed]
- Le Pichon JB, Riordan SM, Watchko J, et al. The Neurological Sequelae of Neonatal Hyperbilirubinemia: Definitions, Diagnosis and Treatment of the Kernicterus Spectrum Disorders (KSDs). Curr Pediatr Rev 2017;13:199-209. [PubMed]
- Amin SB, Smith T, Timler G. Developmental influence of unconjugated hyperbilirubinemia and neurobehavioral disorders. Pediatr Res 2019;85:191-7. [Crossref] [PubMed]
- Bhutani VK, Johnson-Hamerman L. The clinical syndrome of bilirubin-induced neurologic dysfunction. Semin Fetal Neonatal Med 2015;20:6-13. [Crossref] [PubMed]
- Diamond I, Schmid RS. Experimental bilirubin encephalopathy. The mode of entry of bilirubin-14C into the central nervous system. J Clin Invest 1966;45:678-89. [Crossref] [PubMed]
- Hansen TW. Bilirubin Entry into and Clearance from Rat Brain during Hypercarbia and Hyperosmolality. Pediatr Res 1996;39:72-6. [Crossref] [PubMed]
- Hansen TW, Oyasaeter S, Stiris T, et al. Effects of sulfisoxazole, hypercarbia, and hyperosmolality on entry of bilirubin and albumin into brain regions in young rats. Biol Neonate 1989;56:22-30. [Crossref] [PubMed]
- Levine RL, Fredericks WR, Rapoport SI. Clearance of Bilirubin from Rat Brain after Reversible Osmotic Opening of the Blood-Brain Barrier. Pediatr Res 1985;19:1040-3. [Crossref] [PubMed]
- Hegyi T, Kleinfeld A, Huber A, et al. Effects of Soybean Lipid Infusion on Unbound Free Fatty Acids and Unbound Bilirubin in Preterm Infants. J Pediatr 2017;184:45-50.e1. [Crossref] [PubMed]
- Hegyi T, Kleinfeld A, Huber A, et al. Unbound bilirubin levels in phototherapy-treated preterm infants receiving soy-based lipid emulsion. Pediatr Int 2020;62:1357-63. [Crossref] [PubMed]
- Hegyi T, Kathiravan S, Stahl GE, et al. Unbound free fatty acids from preterm infants treated with intralipid decouples unbound from total bilirubin potentially making phototherapy ineffective. Neonatology 2013;104:184-7. [Crossref] [PubMed]
- Strauss KA, Robinson DL, Vreman HJ, et al. Management of hyperbilirubinemia and prevention of kernicterus in 20 patients with Crigler-Najjar disease. Eur J Pediatr 2006;165:306-19. [Crossref] [PubMed]
- Amin SB. Effect of Free Fatty Acids on Bilirubin-Albumin Binding Affinity and Unbound Bilirubin in Premature Infants. JPEN J Parenter Enteral Nutr 2010;34:414-20. [Crossref] [PubMed]
- Robertson A, Fink S, Karp W. Effect of cephalosporins on bilirubin-albumin binding. J Pediatr 1988;112:291-4. [Crossref] [PubMed]
- Amin SB, Harte T, Scholer L, et al. Intravenous Lipid and Bilirubin-Albumin Binding Variables in Premature Infants. Pediatrics 2009;124:211-7. [Crossref] [PubMed]
- Ahlfors CE. Effect of ibuprofen on bilirubin-albumin binding. J Pediatr 2004;144:386-8. [Crossref] [PubMed]
- Groenendaal F, van der Grond J, de Vries LS. Cerebral metabolism in severe neonatal hyperbilirubinemia. Pediatrics 2004;114:291-4. [Crossref] [PubMed]
- Martich-Kriss V, Kollias SS, Ball WS. MR findings in kernicterus. AJNR Am J Neuroradiol 1995;16:819-21. [PubMed]
- Oakden WK, Moore AM, Blaser S, et al. 1H MR spectroscopic characteristics of kernicterus: a possible metabolic signature. AJNR Am J Neuroradiol 2005;26:1571-4. [PubMed]
- Triulzi F, Parazzini C, Righini A. Patterns of damage in the mature neonatal brain. Pediatr Radiol 2006;36:608-20. [Crossref] [PubMed]
- Watchko JF. Kernicterus and the molecular mechanisms of bilirubin-induced CNS injury in newborns. Neuromolecular Med 2006;8:513-29. [Crossref] [PubMed]
- Cannon C, Daood MJ, O’Day TL, et al. Sex-specific regional brain bilirubin content in hyperbilirubinemic Gunn rat pups. Biol Neonate 2006;90:40-5. [Crossref] [PubMed]
- Conlee JW, Shapiro SM. Development of cerebellar hypoplasia in jaundiced Gunn rats: a quantitative light microscopic analysis. Acta Neuropathol 1997;93:450-60. [Crossref] [PubMed]
- Gazzin S, Zelenka J, Zdrahalova L, et al. Bilirubin accumulation and Cyp mRNA expression in selected brain regions of jaundiced Gunn rat pups. Pediatr Res 2012;71:653-60. [Crossref] [PubMed]
- Schutta HS, Johnson L. Clinical signs and morphologic abnormalities in Gunn rats treated with sulfadimethoxine. J Pediatr 1969;75:1070-9. [Crossref] [PubMed]
- Shapiro SM. Somatosensory and Brainstem Auditory Evoked Potentials in the Gunn Rat Model of Acute Bilirubin Neurotoxicity. Pediatr Res 2002;52:844-9. [Crossref] [PubMed]
- Hankø E, Tommarello S, Watchko JF, et al. Administration of Drugs Known to Inhibit P-Glycoprotein Increases Brain Bilirubin and Alters the Regional Distribution of Bilirubin in Rat Brain. Pediatr Res 2003;54:441-5. [Crossref] [PubMed]
- Hansen TWR. Bilirubin in the Brain: Distribution and Effects on Neurophysiological and Neurochemical Processes. Clin Pediatr (Phila) 1994;33:452-9. [Crossref] [PubMed]
- Hansen TWR, Tommarello S, Watchko JF. Inhibition of Blood-brain Barrier (BBB) P-glycoprotein (PGP) Function by Various Drugs Enhances Acute Bilirubin (B) Entry into Brain 1020. Pediatr Res 1998;43:176. [Crossref]
- Hansen T, Tommarello S, Allen J. Subcellular localization of bilirubin in rat brain after in vivo i.v. administration of [3H]bilirubin. Pediatr Res 2001;49:203-7. [Crossref] [PubMed]
- Roger C, Koziel V, Vert P, et al. Effects of bilirubin infusion on local cerebral glucose utilization in the immature rat. Brain Res Dev Brain Res 1993;76:115-30. [Crossref] [PubMed]
- Gambaro SE, Robert MC, Tiribelli C, et al. Role of brain cytochrome P450 mono-oxygenases in bilirubin oxidation-specific induction and activity. Arch Toxicol 2016;90:279-90. [Crossref] [PubMed]
- Mustafa MG, King TE. Binding of Bilirubin with Lipid a possible mechanism of its toxic reactions in mitochondria. J Biol Chem 1970;245:1084-9. [Crossref] [PubMed]
- Brito MA, Brites D, Butterfield DA. A link between hyperbilirubinemia, oxidative stress and injury to neocortical synaptosomes. Brain Res 2004;1026:33-43. [Crossref] [PubMed]
- Rodrigues CMP, Solá S, Castro RE, et al. Perturbation of membrane dynamics in nerve cells as an early event during bilirubin-induced apoptosis. J Lipid Res 2002;43:885-94. [Crossref] [PubMed]
- Barateiro A, Domingues HS, Fernandes A, et al. Rat cerebellar slice cultures exposed to bilirubin evidence reactive gliosis, excitotoxicity and impaired myelinogenesis that is prevented by AMPA and TNF-α inhibitors. Mol Neurobiol 2014;49:424-39. [Crossref] [PubMed]
- Calligaris R, Bellarosa C, Foti R, et al. A transcriptome analysis identifies molecular effectors of unconjugated bilirubin in human neuroblastoma SH-SY5Y cells. BMC Genomics 2009;10:543. [Crossref] [PubMed]
- Fernandes A, Silva RFM, Falcão AS, et al. Cytokine production, glutamate release and cell death in rat cultured astrocytes treated with unconjugated bilirubin and LPS. J Neuroimmunol 2004;153:64-75. [Crossref] [PubMed]
- Giraudi PJ, Bellarosa C, Coda-Zabetta CD, et al. Functional induction of the cystine-glutamate exchanger system Xc(-) activity in SH-SY5Y cells by unconjugated bilirubin. PLoS One 2011;6:e29078 [Crossref] [PubMed]
- Qaisiya M, Coda Zabetta CD, Bellarosa C, et al. Bilirubin mediated oxidative stress involves antioxidant response activation via Nrf2 pathway. Cell Signal 2014;26:512-20. [Crossref] [PubMed]
- Qaisiya M, Brischetto C, Jašprová J, et al. Bilirubin-induced ER stress contributes to the inflammatory response and apoptosis in neuronal cells. Arch Toxicol 2017;91:1847-58. [Crossref] [PubMed]
- Rawat V, Bortolussi G, Gazzin S, et al. Bilirubin-Induced Oxidative Stress Leads to DNA Damage in the Cerebellum of Hyperbilirubinemic Neonatal Mice and Activates DNA Double-Strand Break Repair Pathways in Human Cells. Oxid Med Cell Longev 2018;2018:1801243 [Crossref] [PubMed]
- Rodrigues CMP, Solá S, Brito MA, et al. Bilirubin directly disrupts membrane lipid polarity and fluidity, protein order, and redox status in rat mitochondria. J Hepatol 2002;36:335-41. [Crossref] [PubMed]
- Rodrigues CM, Solá S, Brites D. Bilirubin induces apoptosis via the mitochondrial pathway in developing rat brain neurons. Hepatology 2002;35:1186-95. [Crossref] [PubMed]
- Vodret S, Bortolussi G, Jašprová J, et al. Inflammatory signature of cerebellar neurodegeneration during neonatal hyperbilirubinemia in Ugt1 (-/-) mouse model. J Neuroinflammation 2017;14:64. [Crossref] [PubMed]
- Robert MC, Furlan G, Rosso N, et al. Alterations in the cell cycle in the cerebellum of hyperbilirubinemic Gunn rat: a possible link with apoptosis? PLoS One 2013;8:e79073 [Crossref] [PubMed]
- Brites D. The evolving landscape of neurotoxicity by unconjugated bilirubin: role of glial cells and inflammation. Front Pharmacol 2012;3:88. [Crossref] [PubMed]
- Brites D, Fernandes A, Falcão AS, et al. Biological risks for neurological abnormalities associated with hyperbilirubinemia. J Perinatol 2009;29:S8-13. [Crossref] [PubMed]
- Watchko JF, Tiribelli C. Bilirubin-induced neurologic damage--mechanisms and management approaches. N Engl J Med 2013;369:2021-30. [Crossref] [PubMed]
- Hansen TW. Bilirubin Brain Toxicity. J Perinatol 2001;21:S48-51. [Crossref] [PubMed]
- Vaz AR, Silva SL, Barateiro A, et al. Selective vulnerability of rat brain regions to unconjugated bilirubin. Mol Cell Neurosci 2011;48:82-93. [Crossref] [PubMed]
- Dal Ben M, Bottin C, Zanconati F, et al. Evaluation of region selective bilirubin-induced brain damage as a basis for a pharmacological treatment. Sci Rep 2017;7:41032. [Crossref] [PubMed]
- Barateiro A, Chen S, Yueh MF, et al. Reduced Myelination and Increased Glia Reactivity Resulting from Severe Neonatal Hyperbilirubinemia. Mol Pharmacol 2016;89:84-93. [Crossref] [PubMed]
- Shapiro SM. Bilirubin toxicity in the developing nervous system. Pediatr Neurol 2003;29:410-21. [Crossref] [PubMed]
- Keino H, Sato H, Semba R, et al. Mode of prevention by phototherapy of cerebellar hypoplasia in a new Sprague-Dawley strain of jaundiced Gunn rats. Pediatr Neurosci 1985-1986;12:145-50. [Crossref] [PubMed]
- Sawasaki Y, Yamada N, Nakajima H. Developmental features of cerebellar hypoplasia and brain bilirubin levels in a mutant (Gunn) rat with hereditary hyperbilirubinaemia. J Neurochem 1976;27:577-83. [Crossref] [PubMed]
- Silva RF, Rodrigues CM, Brites D. Rat cultured neuronal and glial cells respond differently to toxicity of unconjugated bilirubin. Pediatr Res 2002;51:535-41. [Crossref] [PubMed]
- Falcão AS, Fernandes A, Brito MA, et al. Bilirubin-induced inflammatory response, glutamate release, and cell death in rat cortical astrocytes are enhanced in younger cells. Neurobiol Dis 2005;20:199-206. [Crossref] [PubMed]
- Falcão AS, Fernandes A, Brito MA, et al. Bilirubin-induced immunostimulant effects and toxicity vary with neural cell type and maturation state. Acta Neuropathol 2006;112:95-105. [Crossref] [PubMed]
- Vaz AR, Delgado-Esteban M, Brito MA, et al. Bilirubin selectively inhibits cytochrome c oxidase activity and induces apoptosis in immature cortical neurons: assessment of the protective effects of glycoursodeoxycholic acid. J Neurochem 2010;112:56-65. [Crossref] [PubMed]
- Falcão AS, Silva RFM, Pancadas S, et al. Apoptosis and impairment of neurite network by short exposure of immature rat cortical neurons to unconjugated bilirubin increase with cell differentiation and are additionally enhanced by an inflammatory stimulus. J Neurosci Res 2007;85:1229-39. [Crossref] [PubMed]
- Fernandes A, Falcão AS, Abranches E, et al. Bilirubin as a determinant for altered neurogenesis, neuritogenesis, and synaptogenesis. Dev Neurobiol 2009;69:568-82. [Crossref] [PubMed]
- Falcão AS, Bellarosa C, Fernandes A, et al. Role of multidrug resistance-associated protein 1 expression in the in vitro susceptibility of rat nerve cell to unconjugated bilirubin. Neuroscience 2007;144:878-88. [Crossref] [PubMed]
- Brito MA, Zurolo E, Pereira P, et al. Cerebellar axon/myelin loss, angiogenic sprouting, and neuronal increase of vascular endothelial growth factor in a preterm infant with kernicterus. J Child Neurol 2012;27:615-24. [Crossref] [PubMed]
- Gazzin S, Berengeno AL, Strazielle N, et al. Modulation of Mrp1 (ABCc1) and Pgp (ABCb1) by Bilirubin at the Blood-CSF and Blood-Brain Barriers in the Gunn Rat. PLoS One 2011;6:e16165 [Crossref] [PubMed]
- Barateiro A, Miron VE, Santos SD, et al. Unconjugated bilirubin restricts oligodendrocyte differentiation and axonal myelination. Mol Neurobiol 2013;47:632-44. [Crossref] [PubMed]
- Rice D, Barone S. Critical periods of vulnerability for the developing nervous system: evidence from humans and animal models. Environ Health Perspect 2000;108:511-33. [PubMed]
- Gazzin S, Dal Ben M, Montrone M, et al. Curcumin Prevents Cerebellar Hypoplasia and Restores the Behavior in Hyperbilirubinemic Gunn Rat by a Pleiotropic Effect on the Molecular Effectors of Brain Damage. Int J Mol Sci 2020;22:299. [Crossref] [PubMed]
- Brites D, Fernandes A. Bilirubin-induced neural impairment: a special focus on myelination, age-related windows of susceptibility and associated co-morbidities. Semin Fetal Neonatal Med 2015;20:14-9. [Crossref] [PubMed]
- Vianello E, Zampieri S, Marcuzzo T, et al. Histone acetylation as a new mechanism for bilirubin-induced encephalopathy in the Gunn rat. Sci Rep 2018;8:13690. [Crossref] [PubMed]
- Maisels MJ. Neonatal hyperbilirubinemia and kernicterus - not gone but sometimes forgotten. Early Hum Dev 2009;85:727-32. [Crossref] [PubMed]
- Olusanya BO, Teeple S, Kassebaum NJ. The Contribution of Neonatal Jaundice to Global Child Mortality: Findings from the GBD 2016 Study. Pediatrics 2018;141:e20171471 [Crossref] [PubMed]
- Shapiro SM. Definition of the clinical spectrum of kernicterus and bilirubin-induced neurologic dysfunction (BIND). J Perinatol 2005;25:54-9. [Crossref] [PubMed]
- Slusher TM, Zamora TG, Appiah D, et al. Burden of severe neonatal jaundice: a systematic review and meta-analysis. BMJ Paediatrics Open 2017;1:e000105 [Crossref] [PubMed]
- National Collaborating Centre for Women’s and Children’s Health (UK). Neonatal Jaundice London: RCOG Press, 2010. Available online: http://www.ncbi.nlm.nih.gov/books/NBK65119/
- Vogel JP, Chawanpaiboon S, Moller AB, et al. The global epidemiology of preterm birth. Best Pract Res Clin Obstet Gynaecol 2018;52:3-12. [Crossref] [PubMed]
- Bhutani VK, Johnson L. Kernicterus in Late Preterm Infants Cared for as Term Healthy Infants. Semin Perinatol 2006;30:89-97. [Crossref] [PubMed]
- Okumura A, Kidokoro H, Shoji H, et al. Kernicterus in preterm infants. Pediatrics 2009;123:e1052-8. [Crossref] [PubMed]
- Usman F, Diala UM, Shapiro SM, et al. Acute bilirubin encephalopathy and its progression to kernicterus: current perspectives. Res Rep Neonatol 2018;8:33-44. [Crossref]
- Watchko JF. Bilirubin-Induced Neurotoxicity in the Preterm Neonate. Clin Perinatol 2016;43:297-311. [Crossref] [PubMed]
- Nam GS, Kwak SH, Bae SH, et al. Hyperbilirubinemia and Follow-up Auditory Brainstem Responses in Preterm Infants. Clin Exp Otorhinolaryngol 2019;12:163-8. [Crossref] [PubMed]
- Johnson L, Bhutani VK, Karp K, et al. Clinical report from the pilot USA Kernicterus Registry (1992 to 2004). J Perinatol 2009;29:S25-45. [Crossref] [PubMed]
- Bhutani VK, Wong RJ. Bilirubin neurotoxicity in preterm infants: Risk and prevention. J Clin Neonatol 2013;2:61. [Crossref] [PubMed]
- American Academy of Pediatrics Subcommittee on Hyperbilirubinemia. Management of hyperbilirubinemia in the newborn infant 35 or more weeks of gestation. Pediatrics 2004;114:297-316. Erratum in: Pediatrics 2004 Oct;114(4):1138. [Crossref] [PubMed]
- Govaert P, Lequin M, Swarte R, et al. Changes in globus pallidus with (pre)term kernicterus. Pediatrics 2003;112:1256-63. [Crossref] [PubMed]
- Kamei A, Sasaki M, Akasaka M, et al. Proton Magnetic Resonance Spectroscopic Images in Preterm Infants with Bilirubin Encephalopathy. J Pediatr 2012;160:342-4. [Crossref] [PubMed]
- Moll M, Goelz R, Naegele T, et al. Are recommended phototherapy thresholds safe enough for extremely low birth weight (ELBW) infants? A report on 2 ELBW infants with kernicterus despite only moderate hyperbilirubinemia. Neonatology 2011;99:90-4. [Crossref] [PubMed]
- Watchko JF, Maisels MJ. The enigma of low bilirubin kernicterus in premature infants: Why does it still occur, and is it preventable? Semin Perinatol 2014;38:397-406. [Crossref] [PubMed]
- Morioka I, Nakamura H, Koda T, et al. Serum unbound bilirubin as a predictor for clinical kernicterus in extremely low birth weight infants at a late age in the neonatal intensive care unit. Brain Dev 2015;37:753-7. [Crossref] [PubMed]
- Hegyi T, Chefitz D, Weller A, et al. Unbound bilirubin measurements in term and late-preterm infants. J Matern Fetal Neonatal Med 2020; Epub ahead of print. [Crossref] [PubMed]
- Amin SB, Ahlfors C, Orlando MS, et al. Bilirubin and serial auditory brainstem responses in premature infants. Pediatrics 2001;107:664-70. [Crossref] [PubMed]
- Ahlfors CE, Amin SB, Parker AE. Unbound bilirubin predicts abnormal automated auditory brainstem response in a diverse newborn population. J Perinatol 2009;29:305-9. [Crossref] [PubMed]
- Amin SB, Saluja S, Saili A, et al. Auditory toxicity in late preterm and term neonates with severe jaundice. Dev Med Child Neurol 2017;59:297-303. [Crossref] [PubMed]
- Amin SB, Wang H. Unbound unconjugated hyperbilirubinemia is associated with central apnea in premature infants. J Pediatr 2015;166:571-5. [Crossref] [PubMed]
- Maisels MJ, Watchko JF, Bhutani VK, et al. An approach to the management of hyperbilirubinemia in the preterm infant less than 35 weeks of gestation. J Perinatol 2012;32:660-4. [Crossref] [PubMed]
- Shapiro SM, Riordan SM. Review of bilirubin neurotoxicity II: preventing and treating acute bilirubin encephalopathy and kernicterus spectrum disorders. Pediatr Res 2020;87:332-7. [Crossref] [PubMed]
- Wolf MF, Childers J, Gray KD, et al. Exchange transfusion safety and outcomes in neonatal hyperbilirubinemia. J Perinatol 2020;40:1506-12. [Crossref] [PubMed]
- Horgan MJ. Management of the late preterm infant: not quite ready for prime time. Pediatr Clin North Am 2015;62:439-51. [Crossref] [PubMed]
- Raju TNK. Developmental physiology of late and moderate prematurity. Semin Fetal Neonatal Med 2012;17:126-31. [Crossref] [PubMed]
- Wallenstein MB, Bhutani VK. Jaundice and kernicterus in the moderately preterm infant. Clin Perinatol 2013;40:679-88. [Crossref] [PubMed]
- Oh W, Stevenson DK, Tyson JE, et al. Influence of Clinical Status on the Association Between Plasma Total and Unbound Bilirubin and Death or Adverse Neurodevelopmental Outcomes in Extremely Low Birth Weight Infants. Acta Paediatr 2010;99:673-8. [Crossref] [PubMed]
- Nakamura H, Yonetani M, Uetani Y, et al. Determination of serum unbound bilirubin for prediction of kernicterus in low birthweight infants. Acta Paediatr Jpn 1992;34:642-7. [Crossref] [PubMed]
- Amin SB, Wang H, Laroia N, et al. Unbound Bilirubin and Auditory Neuropathy Spectrum Disorder in Late Preterm and Term Infants with Severe Jaundice. J Pediatr 2016;173:84-9. [Crossref] [PubMed]
- Amin SB, Saluja S, Saili A, et al. Chronic Auditory Toxicity in Late Preterm and Term Infants With Significant Hyperbilirubinemia. Pediatrics 2017;140:e20164009 [Crossref] [PubMed]
- Amin SB, Charafeddine L, Guillet R. Transient bilirubin encephalopathy and apnea of prematurity in 28 to 32 weeks gestational age infants. J Perinatol 2005;25:386-90. [Crossref] [PubMed]
- Ye H, Xing Y, Zhang L, et al. Bilirubin-induced neurotoxic and ototoxic effects in rat cochlear and vestibular organotypic cultures. Neurotoxicology 2019;71:75-86. [Crossref] [PubMed]
- Shaia WT, Shapiro SM, Spencer RF. The jaundiced gunn rat model of auditory neuropathy/dyssynchrony. Laryngoscope 2005;115:2167-73. [Crossref] [PubMed]
- Riordan SM, Bittel DC, Le Pichon JB, et al. A Hypothesis for Using Pathway Genetic Load Analysis for Understanding Complex Outcomes in Bilirubin Encephalopathy. Front Neurosci 2016;10:376. [Crossref] [PubMed]
- Adamkin DH. Late preterm infants: severe hyperbilirubinemia and postnatal glucose homeostasis. J Perinatol 2009;29:S12-7. [Crossref] [PubMed]
- Gkoltsiou K, Tzoufi M, Counsell S, et al. Serial brain MRI and ultrasound findings: relation to gestational age, bilirubin level, neonatal neurologic status and neurodevelopmental outcome in infants at risk of kernicterus. Early Hum Dev 2008;84:829-38. [Crossref] [PubMed]
- Hankø E, Hansen TWR, Almaas R, et al. Bilirubin induces apoptosis and necrosis in human NT2-N neurons. Pediatr Res 2005;57:179-84. [Crossref] [PubMed]
- Nizam MA, Alvi AS, Hamdani MM, et al. Efficacy of double versus single phototherapy in treatment of neonatal jaundice: a meta-analysis. Eur J Pediatr 2020;179:865-74. [Crossref] [PubMed]
- Yurdakök M. Phototherapy in the newborn: what’s new? Journal of Pediatric and Neonatal Individualized Medicine 2015;4:e040255
- Tyson JE, Pedroza C, Langer J, et al. Does aggressive phototherapy increase mortality while decreasing profound impairment among the smallest and sickest newborns? J Perinatol 2012;32:677-84. [Crossref] [PubMed]
- Arnold C, Pedroza C, Tyson JE. Phototherapy in ELBW newborns: Does it work? Is it safe? The evidence from randomized clinical trials. Semin Perinatol 2014;38:452-64. [Crossref] [PubMed]
- Ramy N, Ghany EA, Alsharany W, et al. Jaundice, phototherapy and DNA damage in full-term neonates. J Perinatol 2016;36:132-6. [Crossref] [PubMed]
- Arnold C, Tyson JE, Pedroza C, et al. Cycled Phototherapy Dose-Finding Study for Extremely Low-Birth-Weight Infants: A Randomized Clinical Trial. JAMA Pediatr 2020;174:649-56. [Crossref] [PubMed]
- Greco C, Arnolda G, Boo NY, et al. Neonatal Jaundice in Low- and Middle-Income Countries: Lessons and Future Directions from the 2015 Don Ostrow Trieste Yellow Retreat. Neonatology 2016;110:172-80. [Crossref] [PubMed]
- Olusanya BO, Ogunlesi TA, Slusher TM. Why is kernicterus still a major cause of death and disability in low-income and middle-income countries? Arch Dis Child 2014;99:1117-21. [Crossref] [PubMed]
- Borden AR, Satrom KM, Wratkowski P, et al. Variation in the Phototherapy Practices and Irradiance of Devices in a Major Metropolitan Area. Neonatology 2018;113:269-74. [Crossref] [PubMed]
- Flaherman VJ, Kuzniewicz MW, Escobar GJ, et al. Total Serum Bilirubin Exceeding Exchange Transfusion Thresholds in the Setting of Universal Screening. J Pediatr 2012;160:796-800.e1. [Crossref] [PubMed]
- Murki S, Kumar P. Blood exchange transfusion for infants with severe neonatal hyperbilirubinemia. Semin Perinatol 2011;35:175-84. [Crossref] [PubMed]
- Sabzehei MK, Basiri B, Shokouhi M, et al. Complications of exchange transfusion in hospitalized neonates in two neonatal centers in Hamadan, a five-year experience. Journal of Comprehensive Pediatrics 2015;6:e20587 [Crossref]
- Geiger AS, Rice AC, Shapiro SM. Minocycline blocks acute bilirubin-induced neurological dysfunction in jaundiced Gunn rats. Neonatology 2007;92:219-26. [Crossref] [PubMed]
- Lin S, Wei X, Bales KR, et al. Minocycline blocks bilirubin neurotoxicity and prevents hyperbilirubinemia-induced cerebellar hypoplasia in the Gunn rat. Eur J Neurosci 2005;22:21-7. [Crossref] [PubMed]
- Vodret S, Bortolussi G, Iaconcig A, et al. Attenuation of neuro-inflammation improves survival and neurodegeneration in a mouse model of severe neonatal hyperbilirubinemia. Brain Behav Immun 2018;70:166-78. [Crossref] [PubMed]
- Valaes T, Petmezaki S, Henschke C, et al. Control of Jaundice in Preterm Newborns by an Inhibitor of Bilirubin Production: Studies with Tin-Mesoporphyrin. Pediatrics 1994;93:1-11. [PubMed]
- Reddy P, Najundaswamy S, Mehta R, et al. Tin-mesoporphyrin in the Treatment of Severe Hyperbilirubinemia in a Very-low-birth-weight Infant. J Perinatol 2003;23:507-8. [Crossref] [PubMed]
- Bhutani VK, Poland R, Meloy LD, et al. Clinical trial of tin mesoporphyrin to prevent neonatal hyperbilirubinemia. J Perinatol 2016;36:533-9. [Crossref] [PubMed]
- Hupe M, Li MX, Kneitz S, et al. Gene expression profiles of brain endothelial cells during embryonic development at bulk and single-cell levels. Sci Signal 2017;10:eaag2476 [Crossref] [PubMed]
- Gazzin S, Strazielle N, Tiribelli C, et al. Transport and metabolism at blood-brain interfaces and in neural cells: relevance to bilirubin-induced encephalopathy. Front Pharmacol 2012;3:89. [Crossref] [PubMed]
- Sweeney MD, Zhao Z, Montagne A, et al. Blood-Brain Barrier: From Physiology to Disease and Back. Physiol Rev 2019;99:21-78. [Crossref] [PubMed]
- Daneman R, Agalliu D, Zhou L, et al. Wnt/beta-catenin signaling is required for CNS, but not non-CNS, angiogenesis. Proc Natl Acad Sci U S A 2009;106:641-6. Erratum in: Proc Natl Acad Sci U S A 2009 Apr 14;106(15):6422. [Crossref] [PubMed]
- Bauer HC, Bauer H, Lametschwandtner A, et al. Neovascularization and the appearance of morphological characteristics of the blood-brain barrier in the embryonic mouse central nervous system. Brain Res Dev Brain Res 1993;75:269-78. [Crossref] [PubMed]
- Daneman R, Zhou L, Kebede AA, et al. Pericytes are required for blood-brain barrier integrity during embryogenesis. Nature 2010;468:562-6. [Crossref] [PubMed]
- Lehtinen MK, Bjornsson CS, Dymecki SM, et al. The Choroid Plexus and Cerebrospinal Fluid: Emerging Roles in Development, Disease, and Therapy. J Neurosci 2013;33:17553-9. [Crossref] [PubMed]
- Saunders NR, Dziegielewska KM, Møllgård K, et al. Influx mechanisms in the embryonic and adult rat choroid plexus: a transcriptome study. Front Neurosci 2015;9:123. [Crossref] [PubMed]
- Coelho-Santos V, Shih AY. Postnatal development of cerebrovascular structure and the neurogliovascular unit. Wiley Interdiscip Rev Dev Biol 2020;9:e363 [Crossref] [PubMed]
- Liddelow SA, Dziegielewska KM, VandeBerg JL, et al. Modification of protein transfer across blood/cerebrospinal fluid barrier in response to altered plasma protein composition during development. Eur J Neurosci 2011;33:391-400. [Crossref] [PubMed]
- 190. Hammarlund-Udenaes M, de Lange E, Thorne R. editiors. Drug Delivery to the Brain: Physiological Concepts, Methodologies and Approaches. Springer Science & Business Media, 2013:737.
- Palmela I, Sasaki H, Cardoso FL, et al. Time-dependent dual effects of high levels of unconjugated bilirubin on the human blood-brain barrier lining. Front Cell Neurosci 2012;6:22. [Crossref] [PubMed]
- Brito MA, Pereira P, Barroso C, et al. New autopsy findings in different brain regions of a preterm neonate with kernicterus: neurovascular alterations and up-regulation of efflux transporters. Pediatr Neurol 2013;49:431-8. [Crossref] [PubMed]
- Xu P, Ling Z, Zhang J, et al. Unconjugated bilirubin elevation impairs the function and expression of breast cancer resistance protein (BCRP) at the blood-brain barrier in bile duct-ligated rats. Acta Pharmacol Sin 2016;37:1129-40. [Crossref] [PubMed]
Cite this article as: Jayanti S, Ghersi-Egea JF, Strazielle N, Tiribelli C, Gazzin S. Severe neonatal hyperbilirubinemia and the brain: the old but still evolving story. Pediatr Med 2021;4:37.